-
-
- Importance in Physics and Engineering
-
- How Do Wings Generate Lift
- Newton’s Third Law and Bernoulli’s Principle
- Thrust and Propulsion Systems
- Helicopter Rotor Dynamics
- Other Designs
-
- Ailerons
- Elevators
- Rudder
- Thrust Reversers and Braking
-
- Lift Is Not Just About Bernoulli
- Interaction of All Newton's Laws
-
- Rocket Propulsion
- Vertical Takeoff and Landing (VTOL) Aircraft
-
- Aircraft Design and Innovation
-
The largest passenger aircraft flying today weighs more than 1.2 million pounds. How does it stay airborne, supported by nothing but thin air?
Lift is the force that aircraft use to counter gravity. Newton’s Third Law is one of the key laws of physics that explains lift.
Let’s see what Newton’s Third Law is, and why we need it to understand lift.
Key Takeaways
- Newton’s Third Law states that for every action, there is an equal and opposite reaction.
- This principle is fundamental in generating lift, thrust, and maneuverability, allowing aircraft to fly.
- Newton’s Third Law helps pilots and engineers improve flight safety and aircraft performance.
- Both Newton’s Third Law and Bernoulli’s Principle contribute to lift generation.
What Is Newton’s Third Law of Motion?
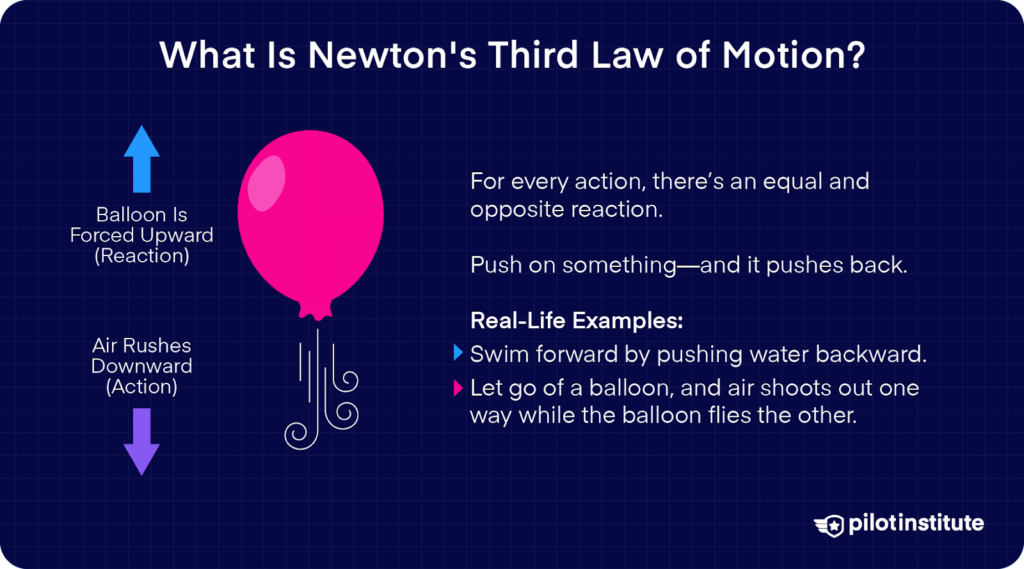
Newton’s laws are one of the cornerstones of classical physics. The third law is one of the simplest and most intuitive laws, even though it has far-reaching consequences.
Newton’s Third Law states that for every action, there is an equal and opposite reaction.
Newton was talking about physical objects. So, if one object pushes on another, the second object pushes back with an equal force in the opposite direction.
Forces always come in pairs – an action and a reaction. This might sound abstract, but it’s something we experience daily.
Think of swimming: you push water backward, and the water pushes you forward.
Let go of a blown-up balloon; the escaping air shoots out one way while the balloon zips off in the other direction.
Importance in Physics and Engineering
Newton’s Third Law is so fundamental in physics and engineering because it tells us there is a consequence for every force we exert on an object.
If we understand it well enough, we can harness this phenomenon to help us even defy gravity.
Developments in aircraft design and engine technology have allowed us to fly farther, faster, and carry more payload. This has only been possible by understanding the laws of physics.
Application of Newton’s Third Law in Aviation
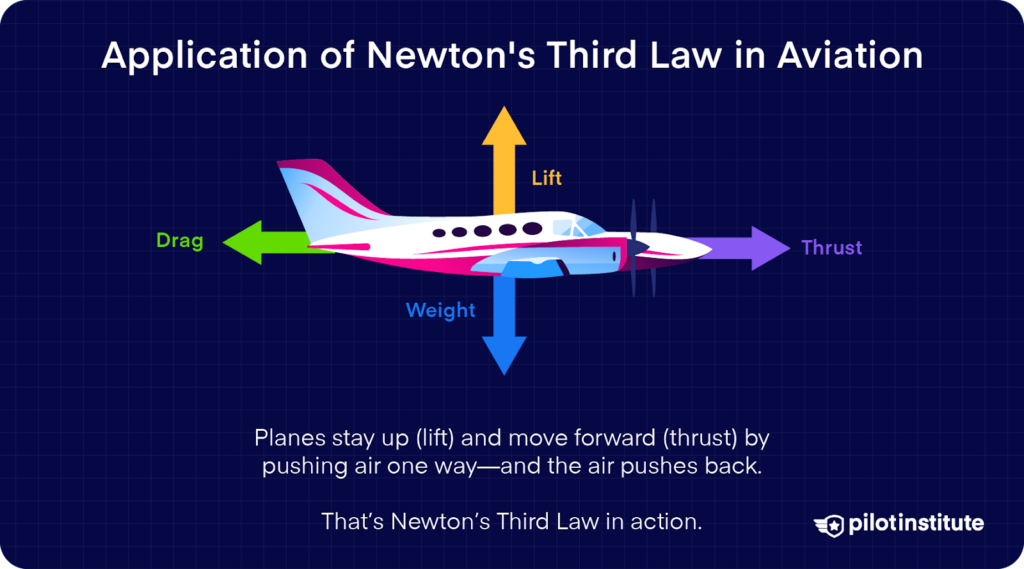
When we talk about airplanes, we focus primarily on two forces:
- Lift (how the aircraft stays aloft).
- Thrust (how it moves forward).
Both rely directly on Newton’s Third Law in the same manner. The aircraft pushes the air in one direction, and the air responds with an equal push on the aircraft in the opposite direction.
Let’s see how each depends on Newton’s Third Law.
How Do Wings Generate Lift
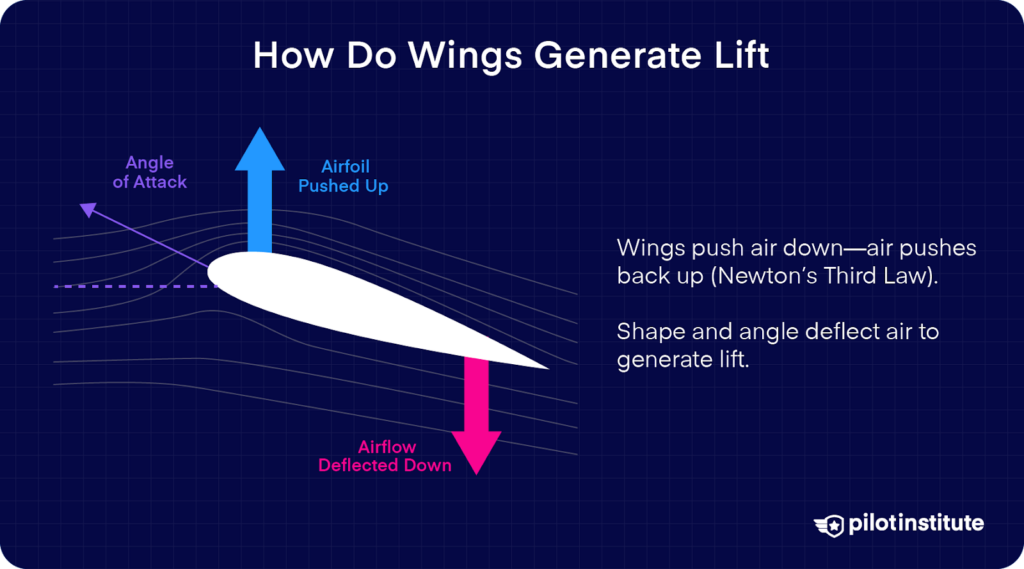
The purpose of wings is to generate lift, that is, create an upward force. Newton’s Third Law tells us that we need to push air downward to produce an upward force.
Wings do just that. They are designed with a special shape called an airfoil, which encourages passing air to turn and deflect downward.
This deflected air is the action. Newton’s Third Law gives us an equal and opposite reaction force on the wing, pushing it upward.
How does the wing manage to deflect the airflow?
Two main factors affect the airflow. One is the upper wing surface’s curvature compared to the lower surface, called wing camber.
High camber generally promotes more airflow deflection, thanks to something called Bernoulli’s Principle. This is why the typical airfoil shape has a curved top and a flat bottom, especially in slower-speed general aviation aircraft.
However, it’s even possible to generate lift from a wing with symmetrical upper and lower surfaces, that is, without camber.
How?
Take a wing with a completely flat upper and lower surface and tilt it so the leading edge is higher than the trailing edge. When the airflow hits this wing, it will meet the leading edge at an angle, curve over the surface, and deflect downwards at the trailing edge.
The angle between the oncoming airflow and a straight line between the leading and trailing edges is called the Angle of Attack (AoA). When the angle of attack is increased, the wing deflects more air downwards.
If the pilot increases the wing’s angle of attack too much, the airflow can separate from the wing’s surface, causing a stall – an abrupt loss of lift.
This angle is called the critical angle of attack.
So, pilots must find the sweet spot: enough angle to generate strong lift but not so steep that airflow breaks away.
Newton’s Third Law and Bernoulli’s Principle
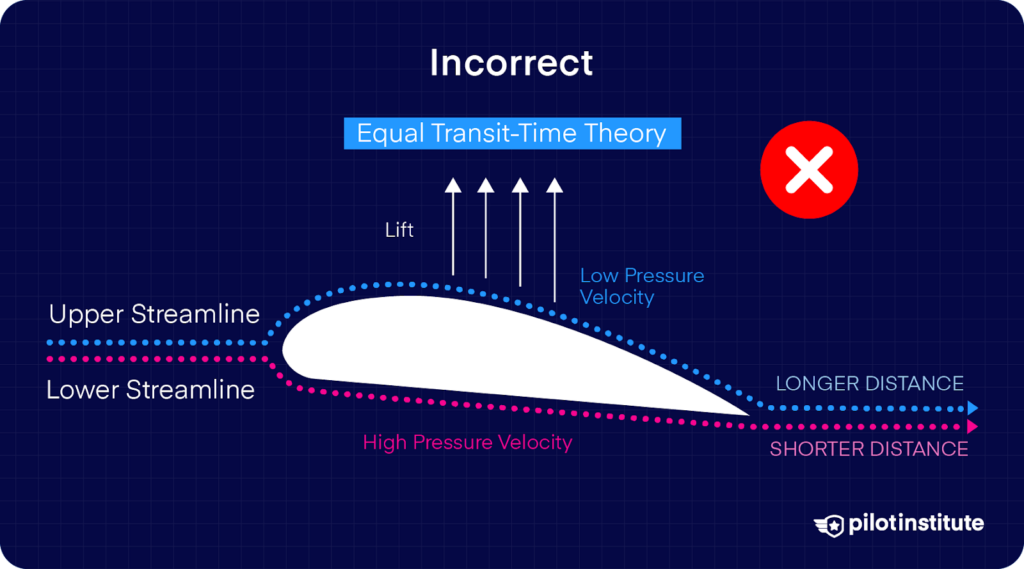
People often answer the question “How do wings create lift?” by saying it’s due to Bernoulli’s Principle. That’s true, but it is only part of the answer.
Bernoulli’s Principle states that as a moving fluid’s velocity increases, the pressure within it decreases. How does that help the wing generate lift?
In the context of aircraft wings, if we increase the airflow velocity above the wing, that area will have low pressure. The air under the wing will have relatively higher pressure. The pressure difference pushes the wing upwards.
That’s the explanation of lift given solely from the Bernoulli Principle perspective.
The problem is that this doesn’t explain how a symmetrical airfoil or even a flat plate can generate lift at high AoA, and yet they do.
At high AoA, Newton’s Third Law – the downward deflection of air – becomes a much more convincing explanation for the lift produced.
You can even see this effect yourself the next time you’re in a moving car. Stick your hand out the window and make a flat ‘wing.’ See what happens when you tilt it upwards. You’ll feel the rushing air hitting your hand and pushing it upwards as it deflects downward, just as the third law predicts.
That’s why an explanation of lift requires Bernoulli’s Principle and Newton’s Third Law to be accurate and complete.
Thrust and Propulsion Systems
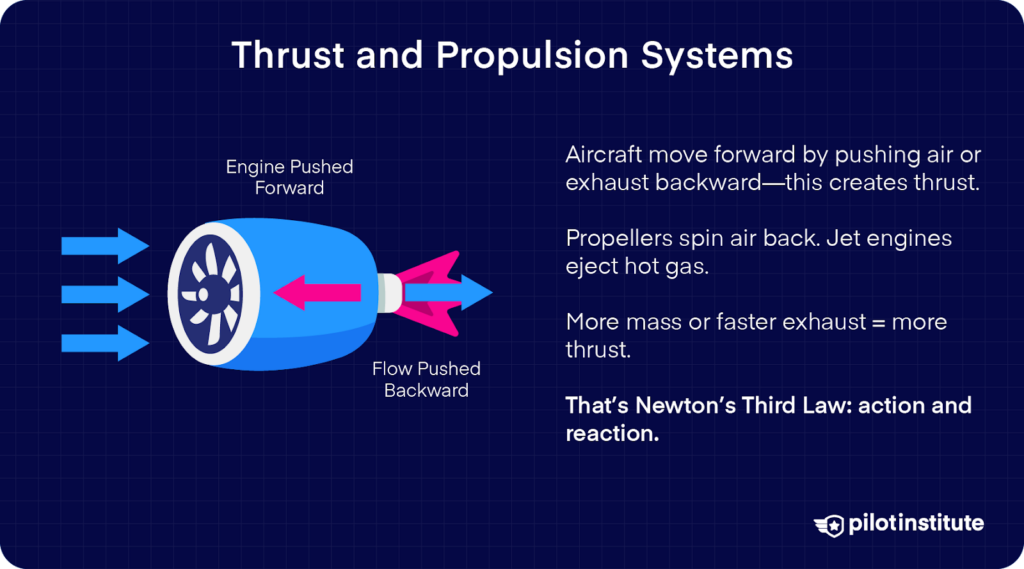
A wing has to move fast enough through the air to generate lift effectively. This means aircraft need to generate a force to push them forward. That force is called thrust.
Thrust relies on Newton’s Third Law as well. The principle is the same whether the aircraft gets thrust from propellers or jet engines.
A propeller forces air backward, while a jet engine ignites a mixture of air and fuel to generate hot gases that eject backward.
Both situations throw mass backward, and the mass pushes the aircraft forward as a reaction.
Let’s clear up a potential misconception here.
In either case, thrust is not generated by the mass pushing against atmospheric air in the sense of a person pushing against a wall to move away.
Simply throwing air or exhaust gases away from the aircraft creates a reaction force that propels the aircraft forward.
This point becomes easier to understand if you consider a rocket in space. There’s no atmospheric air in the vacuum of space, yet rockets still manage to accelerate. How?
The rocket pushes its exhaust gas out the nozzle at high speed (action), and the exhaust gas pushes the rocket forward (reaction). There is nothing “solid” to push on – just momentum exchange.
So, an airplane with a propeller or jet doesn’t need to push on stationary air molecules in the atmosphere; it simply grabs and throws whichever air molecules are available backward.
What does Newton’s Third law tell pilots about thrust?
If you need more thrust, you either need to throw more mass of air backward or throw it faster backward (or both).
That’s exactly what engine controls do.
- In jet engines, increasing throttle increases the fuel flow, producing more exhaust gases that can be flung backward.
- In propellers, increasing the throttle makes the propeller spin faster, flinging air backward harder.
- In variable-pitch propellers, increasing the throttle causes the governor to increase the propeller pitch. The blades bite more air and throw more of it backward.
In each case, the result is increased mass forced backward in a given time. The reaction in the form of increased thrust allows the aircraft to overcome drag and accelerate or climb.
Helicopter Rotor Dynamics
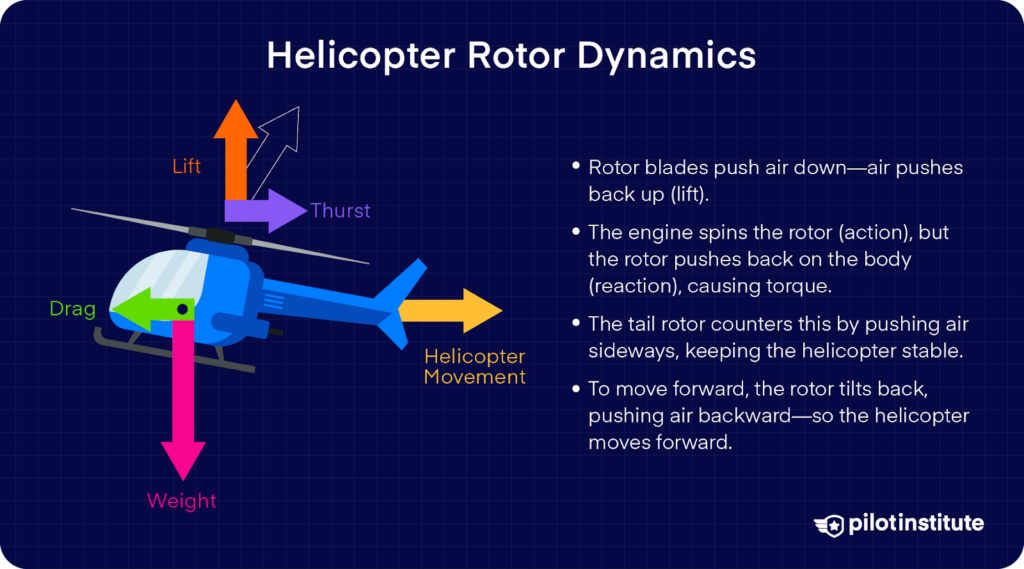
Helicopters might seem very different from airplanes in how they take to the air, but Newton’s Third Law also governs how they fly.
A helicopter’s main rotor has blades, essentially a set of wings, spinning in a circle. As the rotor spins, each blade is an airfoil that forces air downward (action) to produce lift upward (reaction), exactly like an airplane wing.
The pilot’s cyclic and collective controls change the blades’ AoA to control how much lift they generate.
Newton’s Third Law doesn’t stop there. The complication with helicopters is that the main rotor is driven by an engine that applies a twisting force (torque) to spin it.
If the helicopter’s engine twists the rotor blades clockwise (action), the rotor blades exert an equal and opposite twisting force back on the helicopter’s body counter-clockwise (reaction).
This is called torque reaction, and if it’s left unchecked, the helicopter fuselage will spin in the opposite direction of the main rotor. That would not be good for the passengers (nor their stomachs).
Helicopters use a small rotor mounted on the tail that produces sideways thrust to counter the main rotor’s torque reaction. This rotor’s operation is yet another example of Newton’s Third Law. The tail rotor pushes air to one side (action), and the tail moves the other way (reaction).
The pilot controls the amount of this sideways thrust with foot pedals. Pressing a pedal changes the tail rotor blade pitch, increasing or decreasing its thrust to turn the helicopter’s nose.
When a helicopter wants to move forward, the rotor is tilted so that it pushes air back and down. The backward push on the air (action) results in a forward push on the helicopter (reaction).
Other Designs
The tail rotor is not the only way to solve the torque reaction problem.
The torque from a single rotor can be countered using another rotor that spins in the opposite direction. These rotors create equal and opposite torques that cancel out.
Helicopters like the CH-47 Chinook place the two rotors in tandem. Others, such as Russian Kamov models, stack both rotors on each other.
Some designs use ducted fans or even expelled air (NOTAR – NO TAIL Rotor) to counter torque, but the physics principle is the same.
Newton’s Third Law in Aircraft Maneuvers
So far, we’ve looked at how Newton’s Third Law enables lift and forward motion. But it’s equally important in how pilots control and maneuver an aircraft.
Pilots steer aircraft by moving control surfaces. These hinged panels on the wings and tail can redirect airflow if deflected from their neutral position.
Each control surface action is essentially an application of Newton’s Third Law. When a control surface moves, air deflection (action) generates a force on the airplane in the opposite direction (reaction).
Let’s break down the main control surfaces and how they use action-reaction:
Ailerons
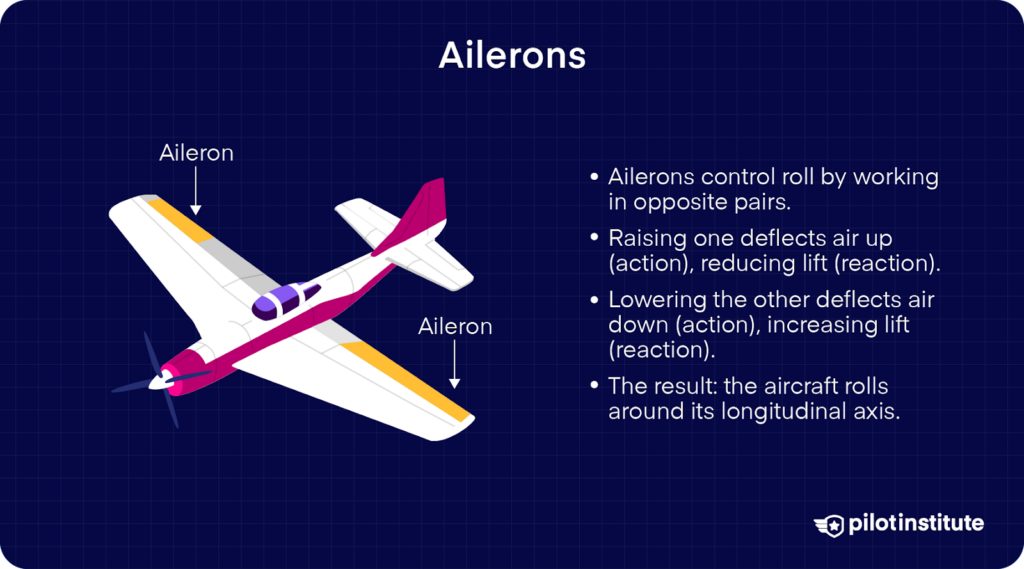
Ailerons are flaps placed on the wings’ trailing edges. They allow roll control about the aircraft’s longitudinal axis. They work in opposite pairs: when one aileron goes up, the other goes down.
If you want to roll the aircraft to the right, you can command the right-wing aileron to rise and the left-wing aileron to lower.
The raised aileron on the right wing deflects airflow upward (action). The downward force on the right wing (reaction) reduces its lift.
Meanwhile, the left wing’s lowered aileron deflects air downward more than usual (action), increasing lift on that side (reaction).
Both forces complement each other, and the net torque about the longitudinal axis makes the aircraft roll.
Elevators
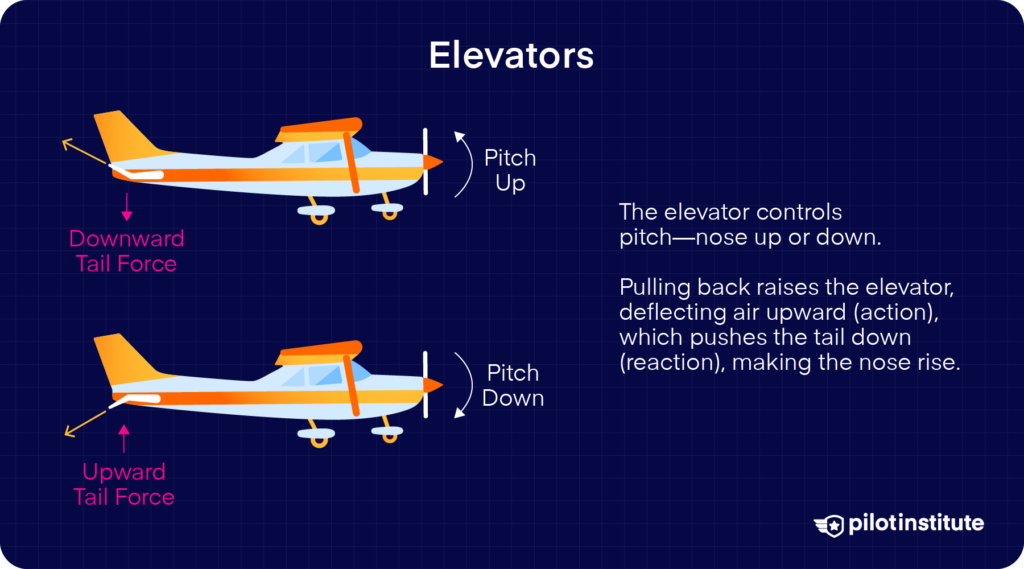
The elevator is the hinged panel on the trailing edge of the aircraft’s horizontal tail. It offers pitch control, meaning the aircraft pivots about the lateral axis. In simple terms, elevators let you raise or lower the aircraft’s nose.
The elevator raises if you pull back on the stick or yoke. This causes the tail to force air upward (action) instead of straight back. This generates a downward force on the tail (reaction).
The aircraft pivots about its center of gravity. The nose tilts upwards as the tail moves down, and the aircraft climbs.
Rudder
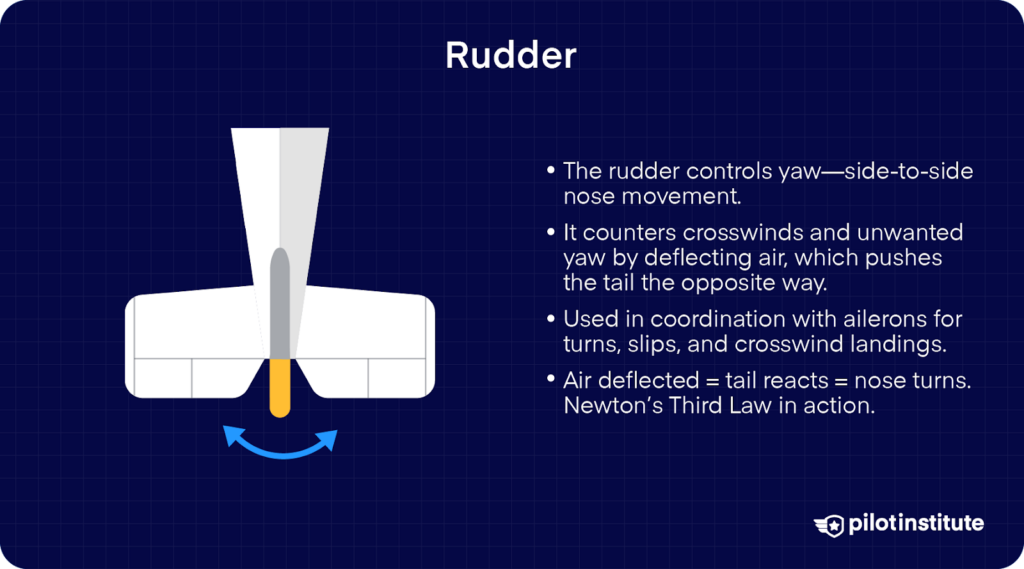
The rudder is the panel on the vertical part of the aircraft’s tail. It provides yaw control. Yaw means side-to-side movement about the vertical axis.
Whenever aircraft encounter crosswinds, the most noticeable impact is felt in yaw. That’s because the tail has a large vertical surface that catches a lot of crosswind. If you don’t do anything to counter it, the impact will push the tail around and swing the aircraft off course.
This is where the rudder comes in. If you press the left pedal, the rudder turns to the left. Oncoming airflow hits the protruding rudder and deflects to the left (action). The impact pushes the tail in the opposite direction towards the right. The aircraft pivots about the vertical axis, and the nose swings to the left.
You’ll often use the rudder to prevent unwanted yaw. Unwanted yaw can come from crosswinds, as mentioned earlier, or from the ailerons as adverse yaw.
A touch of rudder just as you enter a turn, for example, counters adverse yaw and helps keep the aircraft coordinated.
There are even maneuvers where you use the rudder to enter the aircraft into a slip.
You can execute a forward slip by applying rudder in one direction and ailerons in the other. This lets you lose altitude rapidly without speeding up. It’s very useful if you’re too high on approach.
A sideslip is one method to counter crosswinds while on approach. You use the rudder here to align yourself with the runway as the ailerons bank the aircraft into the wind.
In all these cases, the pilot controls the airplane by using the ailerons, elevator, and rudder to push air around, with the air pushing back on the airplane.
By understanding this, pilots can better grasp why the airplane responds as it does.
Thrust Reversers and Braking
Newton’s Third Law is also involved in helping aircraft slow down.
Spoilers are panels on the top of wings that pilots can raise to “spoil” lift and increase drag. When a spoiler pops up into the airflow, it does a couple of things.
- First, it disrupts the smooth airflow, reducing the wing’s lift.
- Secondly, the spoiler itself deflects air upward. The spoiler forces air upwards and slightly forward (action), and the air responds by pushing the wing downward (reaction) and the aircraft backward due to drag.
Both these actions are very useful in the landing roll since the goal is to slow the aircraft down and make it stick to the runway to make the wheel brakes more effective.
Jet aircraft often have thrust reversing mechanisms built into their engines. This allows them to redirect the engine’s thrust forward (action). The mass of air and exhaust gases thrown ahead push back on the aircraft (reaction), effectively increasing drag.
Common Misconceptions
Lift Is Not Just About Bernoulli
Many websites, videos, and even textbooks continue to answer how lift is created by only mentioning Bernoulli’s Theorem. This isn’t wrong, but it’s not the complete truth either. We’ve previously explained Newton’s Third Law’s role in lift generation.
Misconceptions such as the Equal Transit Time myth or the half-venturi theory are convincing because parts of these theories are technically correct.
In reality, the wing does drive air downward. Even a flat plate angled correctly can produce lift – which you can’t explain by curved surfaces alone.
Interaction of All Newton’s Laws
You should also avoid thinking Newton’s Third Law is the only one that matters regarding flight. The first and second laws are definitely involved as well.
Newton’s First Law (inertia) tells us an airplane will keep moving in a straight line at constant speed unless acted on by a net force.
Newton’s Second Law tells us that force is the product of a mass and its acceleration, which explains how much lifting force the mass of air can provide.
The Third Law, as we know, explains why the force acts on the aircraft.
It’s clear that all three laws interact, and their combined effect explains how lift keeps aircraft aloft.
Real-World Examples and Applications
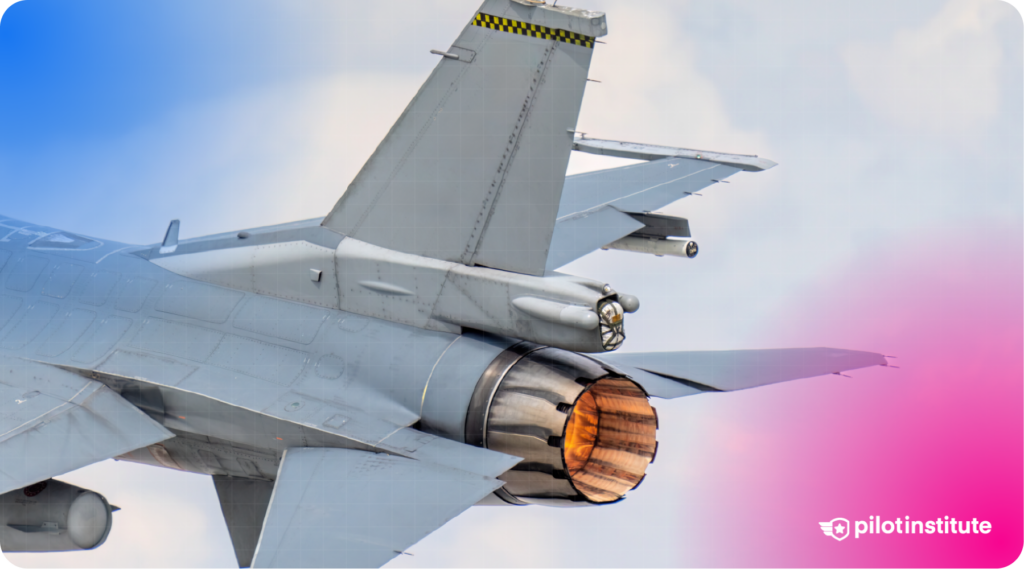
Rocket Propulsion
Rockets operate on Newton’s Third Law so directly that it’s often called the “rocket principle.”
A rocket engine is essentially a device that throws mass (hot gas from burning propellant) in one direction (action) so that the rocket moves in the opposite direction (reaction).
Aircraft engines burn fuel by taking atmospheric air as they fly through it. Rocket engines, operating in a vacuum, have to carry their own oxidizer to get the fuel to burn in a vacuum.
Unlike airplanes, rockets don’t rely on aerodynamics (at least not for the main thrust – they do for steering a bit within the atmosphere).
In the vacuum of space, they work entirely on the principles of action and reaction.
While aircraft maneuver by manipulating passing airflow, objects in space do not have that luxury. That’s why space vehicles use small rockets or thrusters for even minor maneuvering.
Rockets also demonstrate Newton’s First Law. Once coasting in space with engines off, they keep moving until another force acts. Newton’s Second Law dictates the amount of thrust force they need to achieve certain accelerations.
New suborbital spaceplanes such as SpaceShipTwo combine aircraft and rocket principles. After being launched from a carrier aircraft, they use a rocket engine to reach space. After returning to the Earth’s atmosphere, they use wings to glide back to a runway.
Vertical Takeoff and Landing (VTOL) Aircraft
Some aircraft, like the Harrier or F-35B, have adjustable exhaust nozzles. Pointing the exhaust downwards allows them to take off vertically or hover, practically making them operate like rockets.
The engines blast hot exhaust downwards (action), pushing the jet upwards (reaction). No wings required at all.
Once airborne, they can tilt the exhaust nozzles rearward to fly like a normal aircraft. In this mode, the exhaust doesn’t contribute to vertical force, so wings are required to generate lift.
Many helicopter-like drones or craft use multiple small rotors ( essentially propellers pointing up) to achieve VTOL flight. They all rely on the same physics: push air down (action), air pushes you back up (reaction).
We’ve recently seen rockets and spacecraft such as SpaceX’s Falcon 9 and Starship land themselves tail first using engines to slow the descent and touch down gently. This is, again, Newton’s Third Law in action.
VTOL craft and rockets make it clear that flight is possible whenever you generate an upward force greater than weight. You can do it via aerodynamics with wings deflecting air downwards or thrust pushing exhaust gases down.
Importance for Pilots and Engineers
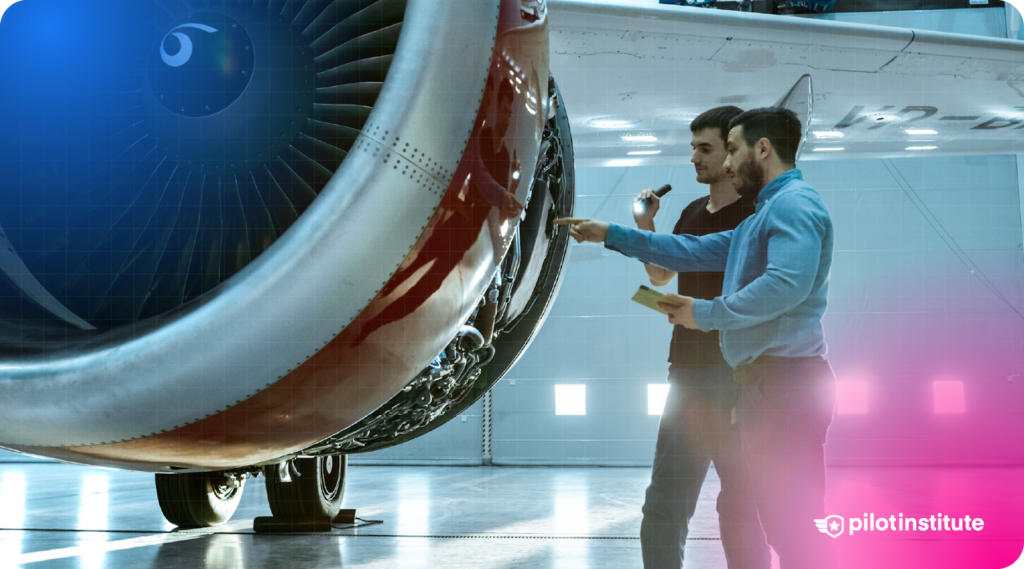
Understanding Newton’s Third Law is very beneficial for pilots. It helps to understand concepts such as stalls and why its recovery procedure requires pushing the nose down.
It will also help you understand propeller effects or why you might need right rudder when taking off in a single-engine propeller aircraft.
Many advanced principles of flight and aerodynamic theories are built on the basis of Newton’s Third Law. Only if you grasp Newton’s Third Law and its implications can you be in a position to understand concepts like P-factor and spiraling slipstream.
Aircraft Design and Innovation
Engineers must understand physics concepts well to design equipment capable of harnessing them.
Newton’s Third Law is so fundamental for physics that it applies to nearly all aspects of aircraft design. For example:
- Thrust generation and propeller design: Optimizing engine thrust and increasing propeller blade efficiency.
- Structural analysis: Reducing structural weight while keeping it strong enough to withstand stresses.
- Aerodynamic design: Designing devices like blended winglets to increase efficiency.
- Material and fatigue analysis: Developing new materials for aircraft construction, such as carbon composites.
- Experimental and hypersonic flight: Understanding extreme reaction forces from shock waves in supersonic conditions.
Conclusion
Newton’s Third Law is fundamental when it comes to aviation. While it is thankfully easy to understand, what’s harder is understanding the implications it presents.
This law helps understand what lifts wings, propels rockets, stabilizes helicopters, steers jets, and even helps bring the aircraft to a stop.
Now that you know the Newtonian explanation, try to understand it from Bernoulli’s perspective.